Electrical properties and structural optimization of GaN/InGaN/GaN tunnel junctions grown by molecular beam epitaxy
1. Introduction
Tunnel junctions (TJs) provide a promising method for the hole injection of p-type GaN. Several research groups have reported preliminary studies of TJs[1−5]. In reverse biased TJs, electrons in the valence band of p-type materials tunnel into the conduction band of n-type materials. Efficient tunneling junctions in Ⅲ-nitride devices can help achieve new device structures and also enhance the performance of electronic and optoelectronic devices[6−10]. However, the low efficiency of the interband tunneling process hinders the widespread application of TJs in electronic and optoelectronic devices. Polarization engineering can overcome the inherent obstacles of these materials. This is done by using the interfacial polarization of the GaN/AlGaN/GaN[11, 12] or GaN/InGaN/GaN[6, 13, 14] junction to induce the high electric field so that the valence band and conduction band on both sides of the junction are aligned. When the InGaN barrier with a lower band gap is used, the decrease of the depletion width and the decrease of the energy barrier results in the high reverse current density[15] of the GaN-based tunnel junction and the tunneling characteristics of the negative differential resistance[16, 17].
Efforts are also being made to develop tunnel junctions grown by metal–organic chemical vapor deposition (MOCVD)[10, 18, 19], and low-resistance TJ has been achieved using InGaN layers with high In content (40%)[20]. However, the use of an InGaN insert layer with a higher component will lead to the deterioration of material quality, strain management and other problems. The use of the high-component InGaN insert layer in TJs remains the main challenge in MOCVD growth. Mg activation is required, which is a big challenge for tunnel junctions grown by MOCVD. The top of a typical tunnel junction used in optoelectronic devices is covered by an n-type layer. It has been reported that hydrogen can only pass through the p-type layer, but not the n-type layer[21, 22]. It requires very complex technological means to activate Mg by mesas etching and thermal annealing[18].
In this work, the InGaN films and GaN/InGaN/GaN TJs were grown on GaN templates by plasma-assisted molecular beam epitaxy (MBE). There is no memory effect of Mg grown by MBE and annealing process after growth is not required since Mg is not passivated by hydrogen. We characterized the properties of InGaN films and we have systematically investigated the effect of the InGaN insert layer in TJs on device electrical properties, in which the InGaN insert layers was various in In composition, InGaN thicknesses and doping concentration. Our results show that GaN/InGaN/GaN TJs can promote good interband tunneling and significantly reduce operating voltage when doping sufficiently large. These low-resistance TJs can be applied to high-power LEDs and laser diodes.
2. Experiment
The samples in this article were grown with the GEN-20A MBE system. The GEN-20A MBE system is a plasma-assisted MBE using a plasma nitrogen source. Other sources such as In, Ga, Mg, and Si are all elemental sources. The N2 flux and radio-frequency plasma power used during the growth were 1.5 sccm and 330 W, respectively. We first grew three single-layer InGaN samples with different In contents on the GaN templates, named S1, S2, and S3 according to the In content from low to high. After determining the single-layer InGaN, we prepared a device containing GaN/InGaN/GaN tunnel junctions, and all structural parameters are shown in
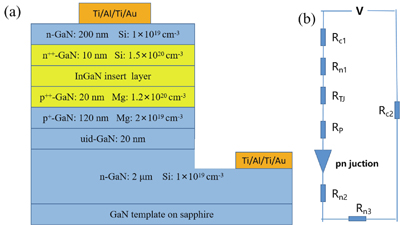
Fig. 1. (Color online) (a) Schematic diagram of the device structure including GaN/InGaN/GaN TJ. (b) Equivalent circuit diagram of the device.
Table 1. The specific structural parameters of GaN/InGaN/GaN TJ.
|
The samples were processed by lithography techniques. Firstly, photoetching a window is required by etching. Secondly, etching mesa by ion beam etching equipment. Thirdly, photoetching metal windows on the mesa and under the mesa. Lastly, depositing metal, wherein the contact metal on the mesa and under the mesa is n-type ohmic contact, and the metal of each layer is classified as Ti/Al/Ti/Au. The In content of the InGaN layers was calibrated by X-ray diffraction (XRD). The surface morphology of the InGaN layers was characterized by atomic force microscope (AFM). The doping concentrations of Mg and Si were calibrated by secondary ion mass spectroscopy (SIMS) on separate doping calibration growth stacks. The interface at the tunnel junction was characterized by high-resolution transmission electron microscopy (HRTEM).
3. Results and discussion
As mentioned earlier, we grew three bulk layer InGaN films on GaN templates, named S1, S2, and S3 according to the In content from low to high. The XRD
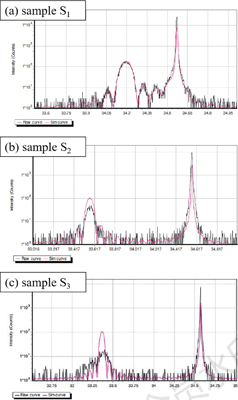
Fig. 2. (Color online) XRD −2θ patterns of the three samples (a) S1 , (b) S2 , (c) S3. The black curve represents the experimental curve, while the red curve represents the simulation curve.
To study the effect of Mg and Si doping concentrations in TJs on device I–V characteristics, we prepared samples A and B. The doping concentration of p++-GaN/p++-InGaN/n++-GaN in sample A is 1 × 1020 cm−3, 1 × 1020 cm−3, and 5 × 1019 cm−3 respectively, and the corresponding doping concentration in sample B is 1.2 × 1020 cm−3, 6 × 1020 cm−3, and 1.5 × 1020 cm−3, respectively. The I–V curves of the samples are shown in

Fig. 5. (Color online) (a) I–V curves of the samples, the doping concentration at the TJ of the samples varies, and (b) the change of differential resistance at forward current density.
The change of differential resistance at forward current density is shown in
To study the effect of In content in the InGaN insert layer on device I–V characteristics, we prepared sample B, C, D and E. The In content of the InGaN insert layer was designed to be 10% (sample B), 25% (sample C), 35% (sample D), and 0% (sample E, no InGaN layer). The I–V curves of the four samples are shown in
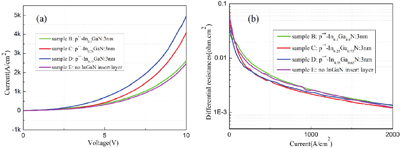
Fig. 6. (Color online) (a) I–V curves of the samples, In content of the InGaN layer at the tunnel junction changes, (b) the change of differential resistance at forward current density.
To study the effect of thickness of In0.35Ga0.65N insert layer in TJ on device I–V characteristics, we prepared sample E, sample F and sample G. The thickness of the In0.35Ga0.65N insert layer was 0 (sample E, no InGaN layer), 3 (sample F), and 5 nm (sample G). The I–V curves of the samples are shown in

Fig. 7. (Color online) I–V curves of the samples, thickness of InGaN layer at tunnel junction varies.
To study the effect of doping in the In0.35Ga0.65N insert layer on device I–V characteristics, we prepared sample F and sample H. The InGaN layer in sample F was not doped, and the InGaN layer in sample H was doped with Si with a concentration of 2 × 1019 cm−3. The I–V curves of the samples are shown in
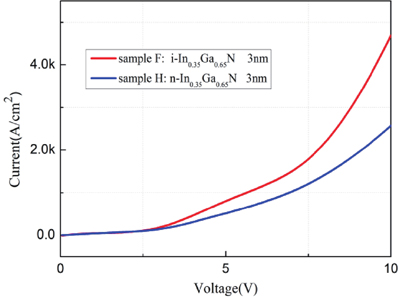
Fig. 8. (Color online) I–V curves of the samples, doping in InGaN layers at tunnel junctions changes.
4. Conclusion
In summary, the InGaN films and GaN/InGaN/GaN tunnel junctions (TJs) were grown on GaN templates by plasma-assisted MBE. As the In content increases, the quality of InGaN films grown on GaN templates decreases and the surface roughness of the samples increases. V-pits and trench defects were not found in the AFM images. p++-GaN/InGaN/n++-GaN TJs were investigated for various In content, InGaN thicknesses and doping concentration in the InGaN insert layer. InGaN insert layer can promote good interband tunneling in GaN/InGaN/GaN TJs and significantly reduce operating voltage when doping is sufficiently high. The current density increases with increasing In content for 3 nm InGaN insert layer, which is achieved by reducing the depletion zone width and the height of the potential barrier. At a forward current density of 500 A/cm2, the measured voltage was 4.31 V and the differential resistance was measured to be 3.75 × 10−3 Ω·cm2 for the device with the 3 nm p++-In0.35Ga0.65N insert layer. When the thickness of the In0.35Ga0.65N layer is closer to the “balanced” thickness, the TJ current density is higher. If the thickness is too high or too low, the width of the depletion zone will increase and the current density will decrease. The undoped InGaN layer has better performance than n-type doping in TJs. Polarization-engineered tunnel junctions can enhance the functionality and performance of electronic and optoelectronic devices.
5 Acknowledge
This work was supported by the National Key Research and Development Program of China (2017YFE0131500, 2022YFB2802801), the National Natural Science Foundation of China (61834008, U21A20493), the Key Research and Development Program of Jiangsu Province (BE2020004, BE2021008-1), and the Suzhou Key Laboratory of New-type Laser Display Technology (SZS2022007). The authors are grateful for the technical support for Nano-X from the Suzhou Institute of Nano-Tech and Nano-Bionics, Chinese Academy of Sciences (SINANO), Chinese Academy of Sciences.
Jun Fang, Fan Zhang, Wenxian Yang, Aiqin Tian, Jianping Liu, Shulong Lu, Hui Yang. Electrical properties and structural optimization of GaN/InGaN/GaN tunnel junctions grown by molecular beam epitaxy[J]. Journal of Semiconductors, 2024, 45(1): 012503.