22 kW near-diffraction-limited Yb:YAG slab laser amplifier without adaptive optics correction
1. Introduction
Lasers with high average power and beam quality are promising sources for materials processing, scientific research, and military defense[1–4]. The conduction-cooled end-pumped slab (CCEPS) configurations have the potential to provide high average power and high beam quality because the zigzag propagation in CCEPS eliminates the thermo-optical path difference (OPD) over the entire beam area[5–9]. Northrop Grumman Corporation, using Nd:YAG CCEPS, first realized more than 100 kW output power in 2009[10,11]. However, the 100 kW laser system with more than 30 slab gain modules (SGMs) was complex and lacked robustness due to the limitation of power extraction from a single SGM.
Yb:YAG is a promising alternative to Nd:YAG for high-average power operation because of its smaller quantum defect and simple electronic structure, resulting in lower volumetric heat generation. In 2001, Goodno et al.[12] first demonstrated a 3-mm-width Yb:YAG CCEPS laser, emitting a power of 252 W with beam quality factors () below 1.5. In 2006, Lu et al. presented a corner-pumped Yb:YAG slab laser that produced a power of 530 W with of 4.3 in a zigzag direction[13]. However, the quasi-three-level nature of Yb:YAG at room temperature makes the lasing threshold high. Under intense pumping and input power, the aggressive thermal effects, such as thermal-induced wavefront distortion and surface damage, limit the output power and degrade the output beam quality. In 2016, Chen et al. reported a MOPA configuration based on a 10-mm-width Yb:YAG CCEPS, which achieved an extraction power of 2.8 kW, but the beam quality was not measurable due to the poor OPD[14]. To simultaneously achieve high average power and high beam quality output, the adaptive optics (AO) system was employed for wavefront correction to improve the beam quality. In 2018, Xu et al. first demonstrated a two-stage Yb:YAG CCEPS MOPA chain with the AO system, obtaining 11.9 kW output power with the beam quality () improved from 9.8 to 2.8. The 30-mm-width SGM in the main amplifier was extracted with a maximum power of 8.3 kW[15]. In the same year, Wang et al. reported a three-stage Yb:YAG CCEPS MOPA chain with an AO system, realizing 22.3 kW output with enhanced from 9.2 to 3.3. The second 30-mm-width SGM in the main amplifier was extracted with a maximum power of 11.3 kW[16]. In 2020, Huang et al. used a four-stage Yb:YAG slab AO-controlled MOPA system, obtaining 21.2 kW laser with a horizontal beam quality (HBQ) improved from 4.2 to 1.9. The single SGM was extracted with a maximum power of 5.3 kW[17]. Although the output performance of AO-controlled Yb:YAG slab laser amplifiers is excellent, the addition of a downstream AO system increases the complexity of laser systems.
In this paper, we experimentally establish a two-stage Yb:YAG CCEPS MOPA chain without an AO system at room temperature, simultaneously achieving an output power of 22 kW and an average beam quality () of 2.95 in 0.5 min. To our knowledge, this is the brightest slab laser without the closed-loop AO system demonstrated to date. By improving the surface quality of the slab and the pumping uniformity of the long-distance transmission within the slab, the SGM in the main amplifier is extracted to 17 kW output power with 33% optical extraction efficiency, as well as less than 0.5 µm OPD of single-pass through the slab. In addition, the power extracted from the SGM is further increased by enlarging the width of the slab. With the improved SGM, the high-power laser system could become simpler and more robust.
2. Experimental Setup
The experimental setup of a two-stage Yb:YAG slab MOPA chain is shown schematically in Fig. 1. A Yb-doped fiber laser, a Yb:YAG CCEPS preamplifier, and a main amplifier form the main parts of the MOPA chain, making it power-scalable.
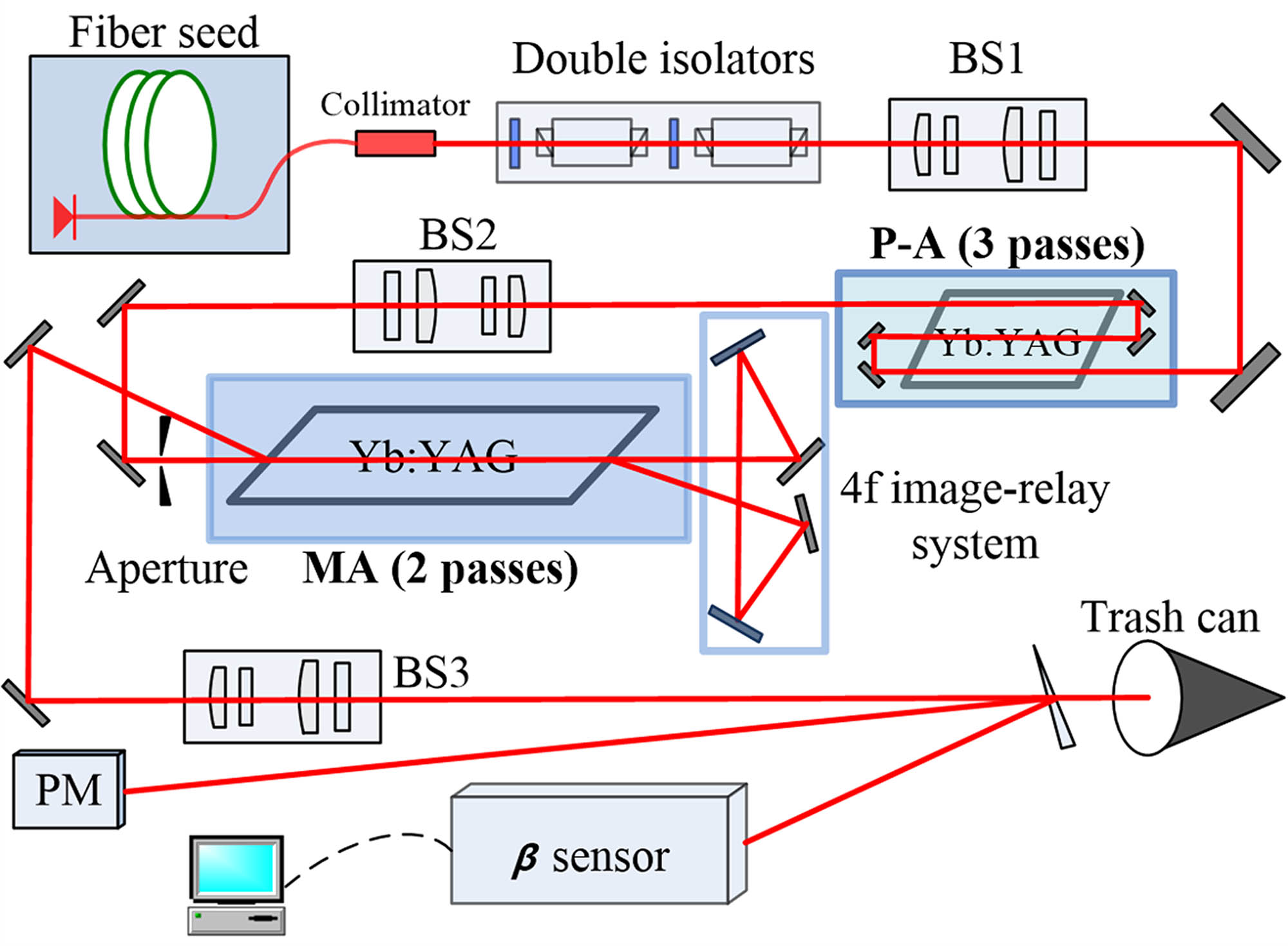
Fig. 1. Experimental setup of Yb:YAG slab MOPA chain. P-A, preamplifier; MA, main amplifier; BS, beam shaper; PM, power meter.
The seed of the MOPA chain is the high-power fiber laser with an output power of 1 kW, a beam quality () of 1.3, a wavelength of 1030 nm, and a linewidth (FWHM) of 0.3 nm. To protect the fiber laser from feedback, the fiber laser was designed as a linear polarization output with an extinction ratio . The beam from the fiber laser was expanded and coupled into the Faraday isolator, and then shaped to by beam shaper 1 (BS1) to match the end profile of the slab in the preamplifier. Based on previous studies[18,19], after three angular and multiplexing passes through the slab in the preamplifier, we obtained a high-brightness preamplifier with an output power of 5 kW and a beam quality () of 1.8. The output beam from the preamplifier is shaped by BS2 to and imaged to match the end profile of the slab in the main amplifier. The high-power inputs to the preamplifier and main amplifier increase the energy storage extraction and reduce the residual gain to suppress the parasitic oscillations. After two angular and multiplexed passes through the slab in the main amplifier, the laser beam was expanded to by BS3 to measure output power and beam quality. In each Yb:YAG slab, the laser propagates in a zigzag pattern. Inside the preamplifier and the main amplifier, the laser propagates through a 4f image relay system. This system consists of two reflective lenses with 1 m focal length (FL). At the exits of the preamplifier, a aperture is positioned to cut the serious aberration part of laser wavefront near the edges of the slab and reduce the backward amplification in the preamplifier. The power loss of the aperture is less than 3%.
Both the SGMs in the preamplifier and main amplifier are the CCEPS configurations. The schematic of a single CCEPS is shown in Fig. 2(a). The pump source is a high-power laser diode array (LDA) with a central wavelength of 940 nm, a spectral width of 5 nm, and a maximum output power of 30 kW. The LDA at each end is delivered by the homogenized waveguide and coupling lens group, and then injected into the slab via TIR from the 45° end faces. The detailed scheme of pump coupling and homogenization system is denoted in Fig. 2(b). A fast axis focusing lens is employed to focus the pump light into the homogenized waveguide. The homogenized waveguide has dimensions of and is made of high purity fused silica (HPFS). The HPFS with OH-concentration of less than 1 ppm (parts per million) reduces the absorption for the 940 nm laser and the thermal effect. After passing through the homogenizing waveguide, the homogenized pump light is shaped and imaged in the slow-axis direction by two cylindrical lenses, called a slow-axis eyepiece with and a slow-axis objective lens with , respectively. Both the cylindrical lenses have an anastigmatic design, which improves the uniformity of long-range transmission within the slab. In fast-axis direction, the homogenized pump light is focused by another cylindrical lens called a fast-axis aspheric lens with . The fast-axis aspheric lens with an aplanatic design enhances the energy concentration to reduce the waste heat at the end face of the slab. A simulation of the pumping light after passing through the homogenizing and coupling system is performed by Zemax. The distributions of the pumping light at the center and entrance of the slab are shown in Figs. 2(c) and 2(d), respectively, which illustrate that the homogeneities of the pumping light are well maintained along the length direction of the slab.

Fig. 2. (a) Schematic of CCEPS gain module; (b) detailed scheme of pump coupling and homogenization system; (c) and (d) distributions of pumping light at the middle of the slab and the entrance, respectively.
The slabs in the preamplifier and main amplifier are multi-concentration-doped, as shown in Fig. 3. The slab of preamplifier is in dimension, while that of the main amplifier is in dimension. The central 62-mm length of the slab is 0.42%-doped Yb:YAG, diffusion-bonded with two 34-mm long, 0.25%-doped Yb:YAG. To reduce the thermal effect and couple pump light into the slab, the rest of the slab is the diffusion-bonded undoped YAG, each of which is 10 mm long. The interfaces between the multi-concentration Yb:YAG slab, constructed by atomic diffusion bonding, have no visible scattered light under 532 nm laser irradiation. The wavefront distortion of slabs is less than . The transmission loss is less than 0.3%/cm, which is the same as the intrinsic loss of YAG. The two end faces of the slab are at a 45° cut. The two edge faces of the slab are roughened and approximately parallel with an angle of 5° to suppress the amplified spontaneous emission (ASE) and parasitic oscillation. The two side faces of the slab are clipped and welded to the microchannel heat sinks for efficient cooling and dissipating heat, mainly produced by the quantum defect of Yb:YAG. Both the heat sinks are cooled by circulating water at the temperature of 20°C. However, each end face and side face with 5-mm-length undoped YAG are not cooled by the heat sinks because of the requirement of coupling the pump light.
For decreasing the thermal effect of the uncooled end caps of the slab in the main amplifier, the surface qualities of the slab are enhanced by the float-polishing (FP) method[20–23]. The defect sizes in the end face of the slab are reduced from 100 µm level to submicrometer level, as shown in Figs. 4(a) and 4(b). Meanwhile, the edge cracks in the stress-concentration regions of the slab, such as the corners and the edges, are dissipated by secondary chamfering and polishing, as shown in Fig. 4(b). Both the 45° end faces are antireflection (AR)-coated at 1030 nm and high-reflection (HR)-coated at 940 nm. The side face has a 3 µm film that is AR-coated at 940 nm and evanescent-wave-coated at 1030 nm. To reduce the thermal effects of the AR and HR films, the absorptivity and structural defects of the films are effectively reduced. The photothermal common-path interferometer (PCI)[24] is used to measure the absorption rate of the films. By optimizing the coating materials, evaporating ion source energy, and rate, the absorption rate of the films is reduced from 300 ppm to less than 20 ppm, as shown in Figs. 5(a) and 5(b).

Fig. 4. Micrograph of the end face of the slab. (a) and (b) 100 µm level and submicrometer level surface, respectively.

Fig. 5. Absorption rate of films. (a) and (b) Maximum absorption rate of 300 ppm and 20 ppm, respectively.
3. Results and Discussion
Under the pump power of 15 kW without laser extraction, the end-face temperature of the main amplifier slab is reduced from 110°C to 40°C by improving the surface quality, as shown in Figs. 6(a) and 6(b). With the temperature reduction, the pump power could be increased, resulting in higher output power from a single slab.
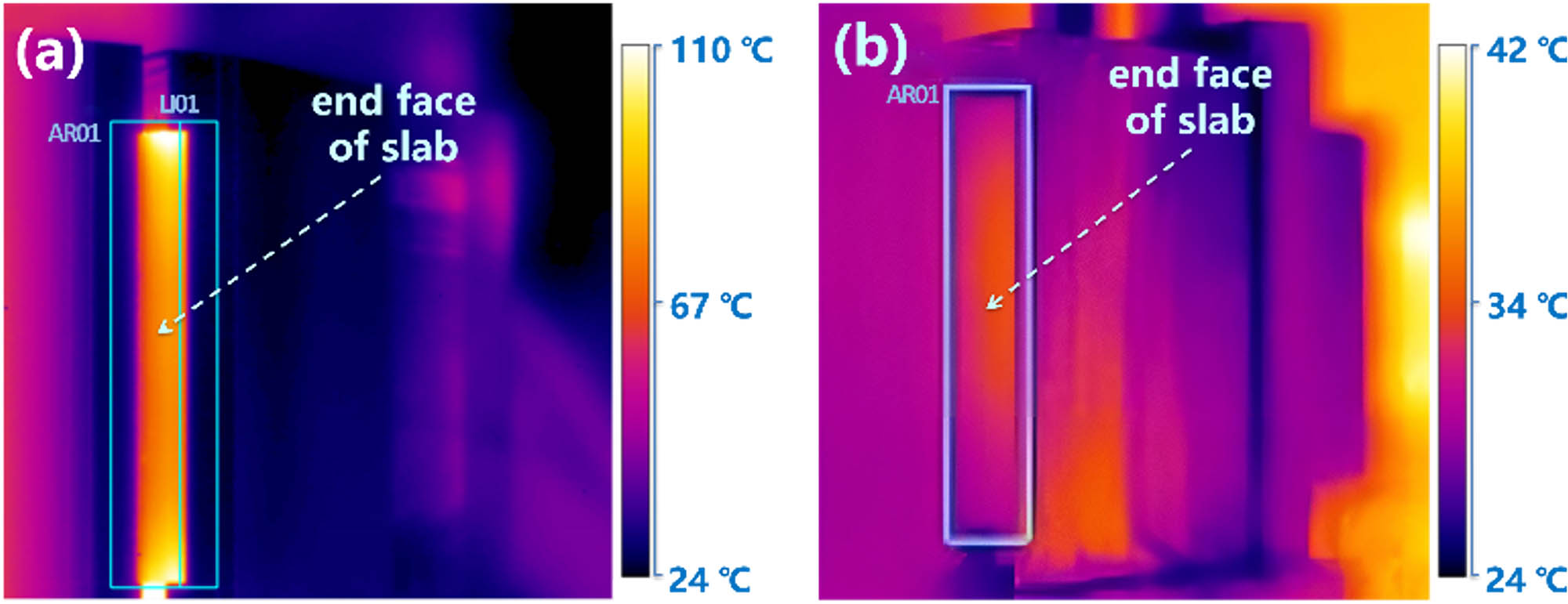
Fig. 6. Temperature comparison of the main amplifier slab end face. (a) and (b) Maximum temperatures of 110°C and 42°C, respectively.
At the input power of 5 kW, the output power of the MOPA chain and the optical extraction efficiency of the main amplifier are shown in Fig. 7(a). Under the pump power of 50 kW, the MOPA chain emits the maximum output power of 22 kW with an optical extraction efficiency of 34%. The optical extraction efficiency is defined here as the ratio of extracted amplifier power to total diode power. The experimental slope efficiency of 45% is achieved by linear fitting, as shown in Fig. 7(b). Based on previous simulation models of quasi-three-level Yb:YAG slab lasers[25–28], we calculate the theoretical output powers. The comparison of the theoretical and experimental dependence of the output power on the pump power is depicted in Fig. 7(b). The theoretical and experimental results show a good agreement, both of which illustrate the output powers could be further scaled linearly, as there are no obvious gain saturation effects.

Fig. 7. (a) Output power of MOPA chain and optical extraction efficiency of main amplifier; (b) comparison of the theoretical and experimental dependence of the output power and slope efficiency.
Benefiting from the improvement of slab surface quality and pumping uniformity, the extraction power of SGM is up to 17 kW, which is much higher than that of other slab lasers. Owing to the CCEPS configuration with the ability of the power scalability by enlarging the width of slab, the power of 42.5 kW could be achieved by using a 10-cm-width slab. With the high-performance SGM, it is possible to reduce the number of slabs in a high-power laser system, making the laser simpler and more robust.
A Shack–Hartman wavefront sensor and a He–Ne laser are used to measure the OPDs of the slab, as shown in Fig. 2(a). The OPD of one passing through the slab at the maximum output power of 22 kW is displayed in Fig. 8(a). In the width direction of the slab, the peak-to-valley (PV) value of the OPD is 5.6 µm. By using quadratic fitting to eliminate defocus, the residual OPD is less than 0.5 µm, depicted in Fig. 8(b). Usually, the influence of defocus on the beam quality could be eliminated by the precompensation approach in experiment. The residual OPD with the low spatial-frequency component in the middle region of the slab indicates little thermal-induced wavefront distortion, leading to a good beam quality of the output beam. Moreover, due to the zigzag propagation compensating the thermal effects, the OPD in the thickness direction is negligible.

Fig. 8. (a) OPD of one passing through the slab at the maximum output power; (b) residual OPD after quadratic fitting to eliminate defocus.
For subsequent laser applications, the output beam of the MOPA chain is expanded to a rectangle of by the BS3. The distribution of the expanded beam in the near field is shown in Fig. 9(a). The aberrations of defocus and tilt in the expanded beam are mostly eliminated by adjusting the distances and orientations between the two lenses in BS3. The beam quality of the expanded beam is measured by a sensor. Under maximum output of 22 kW, the average value of is 2.95 in 0.5 min, for which the minimum is 2.5 while the maximum is 3.3, as shown in Fig. 9(b). The beam profile with beam quality of 2.95 in the far field is exhibited in Fig. 9(c).

Fig. 9. (a) Distribution of the expanded beam in the near field; (b) variation of output beam quality (β) in 0.5 min; (c) far field with the beam quality of 2.95.
4. Conclusion
In this paper, by improving the surface quality of the slab and the uniformity of the pump beam to suppress the thermal effects, the load capacity and wavefront uniformity of the Yb:YAG slab are enhanced. With the SGM, the extraction power of single slab is up to 17 kW, and the residual OPD of a single pass is less than 0.5 µm. The MOPA chain without the AO system emitted an output power of 22 kW with an average of 2.95 in 0.5 min. This is, to the best of our knowledge, the brightest slab laser without AO systems at room temperature demonstrated to date. Compared with the previous 20 kW level slab laser, the reduced number of SGMs and the absence of AO components reduce the complexity of the high-power MOPA chain and improve robustness.
[3] P. Sprangle, B. Hafizi, A. Ting. NRL and the development of the laser weapon system. Future Force Nav. Sci. Technol. Mag., 2015, 2: 18.
[18] Y. N. Wang, T. J. Zhou, J. L. Shang, et al.. Yb slab laser amplifier with a laser output of 7.13 kW, 2 times diffraction limit. Laser Optoelectron. Progress, 2021, 58: 1114007.
[20] Y. Namba, H. Tsuwa. Ultrafine finishing of sapphire single crystal. Ann. CIRP, 1977, 27: 325.
[22] H. G. Gao, J. L. Cao, B. Chen. Float polishing for super smooth surface. Opt. Technique, 1995, 21: 40.
[28] L. Chen, Y. Liu, H. Zhao, et al.. Multiphysics simulation of quasi-three-level end- pumped laser. Laser Infrared, 2020, 50: 58.
Dan Wang, Ping He, Tangjian Zhou, Mi Li, Yingchen Wu, Yanan Wang, Jianli Shang, Qingsong Gao, Kai Zhang, Chun Tang, Rihong Zhu. 22 kW near-diffraction-limited Yb:YAG slab laser amplifier without adaptive optics correction[J]. Chinese Optics Letters, 2024, 22(3): 031402.