基于相变材料的非易失光子多值器件研究
0 引言
人工智能[1-2]的蓬勃发展对计算系统提出了更高的要求,急需发展新的高速度高能效计算硬件。近年来,以Chat GPT为代表的人工智能大模型对算力的需求每3~4个月翻一番[3],当前的人工智能处理硬件主要采用图形处理器(Graphics Processing Unit,GPU),英伟达最先进的图形处理器算力能够达到80 tera FLOPS[4]。以图形处理器为代表的电子计算系统采用冯诺依曼架构,其处理器与存储器分置导致运算过程中频繁搬移数据产生大量的能量消耗,上述所说的图形处理器功耗超过400 W,且电子计算系统中的寄生电容影响了计算速度的进一步提高,无法满足人工智能进一步发展对高速度高能效的计算硬件需求[5-6]。
基于集成光子器件的光子智能加速器快速发展[7-8],为实现高速度高能效的计算硬件提出了一种可行的解决方案。基于马赫-曾德尔干涉仪(Mach-Zehnder Interferometer,MZI)[9-13]的可调多值器件是当前构成光子智能加速器的一种主要技术途径,该类器件具备宽带宽和温度不敏感的优势,使其成为光子硬件加速器的优越选择。但是,该类器件的多值特性是通过热光效应[14-15]和载流子迁移[16-17]来实现调制的,其产生的相移通常都比较小,导致器件的尺寸较大,不利于大规模的集成,同时器件具有易失性,无法长时间保持多值,增加了调制的能量需求。利用相变材料(Phase Change Material,PCM)[18-25]的折射率连续可调且非易失保持的特性,将相变材料与上述器件结合能够实现非易失保持的多值特性,为构建光子智能加速器提供一种新的器件基础。
本文实现了一种基于SbSe相变材料的非易失性多值器件,首先通过Lumerical仿真优化了相变材料长度和厚度等结构参数,在此基础上制备了器件,测试过程中通过对微加热器施加电脉冲对相变材料进行调制,实现了器件的多值特性。
1 器件设计
1.1 结构设计
本文设计的多值器件结构如
在设计过程中,微加热器的热分布对相变材料相变具有重要影响,本文设计了两种不同的微加热器结构并通过COMSOL进行电流和固体传热仿真,验证热量的分布情况。首先,微加热器设计成方块型,如
1.2 结构参数优化
采用Lumerical软件中的2.5D有限元分析(varFDTD)对器件的传输进行了模拟仿真,研究了器件不同参数下的光传输性能。首先,在软件中设置了上述器件结构,从其输入端输入波长1 500 nm~1 600 nm的连续光,在输出端检测光通过率。其次,为了观察相变材料相变过程中器件输出的变化,在仿真中固定相变材料的长度,其在长度方向包含晶化和非晶化两种状态的相变材料,连续改变两者的占比,使其从完全晶化逐渐转变为完全非晶化,观察器件光通过率的变化规律,仿真设置如
分析相变材料长度对器件的影响时,固定相变材料的厚度为30 nm,仿真得到其20 μm~50 μm四个长度器件的通过率变化,1 550 nm波段时结果如
分析相变材料厚度对器件的影响时,固定相变材料的长度为30 μm,仿真得到10 nm~40 nm四个厚度器件的通过率变化。
2 器件制备与测试
2.1 器件制备
第一步准备清洗干净的SOI(顶层硅-氧化层-基底厚度为220 nm-2 μm-675 μm),第二步使用电子束光刻的正胶工艺将波导图形转移到SOI上,用电感耦合等离子体刻蚀(Inductive Coupled Plasma Emission Spectrometer,ICP)的方法形成脊波导,第三步使用紫外光刻负胶工艺将相变材料的图形转移到脊波导上方,用磁控溅射方法在脊波导上沉积SbSe,厚度为30 nm,刚生长制备的SbSe的初始状态为非晶态。然后重复使用紫外光刻和磁控溅射在SbSe上方沉积120 nm厚的ITO,以及在ITO两端形成电极,电极使用10 nm的Cr和120 nm的Au。
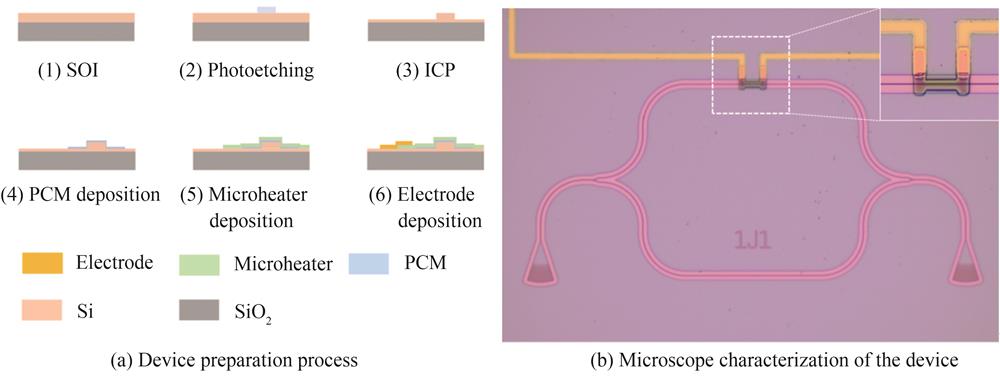
图 5. 器件制备流程及显微镜表征
Fig. 5. Device preparation process and microscope characterization diagram
2.2 多值测试
本实验采用的测试系统分为测试光路与调制电路两部分,如
在测试过程中,相变材料的初始状态为非晶态,为了使相变材料实现晶化,需要施加一个低电压的宽脉冲,使相变材料达到晶化温度并保持一段时间使晶格有序化。对于该器件,施加一个宽度为1 ms,幅度为13.4 V的方形脉冲可以实现晶化。
然而,继续提高电脉冲的能量,由于ITO表面温度与底部温度分布不均匀,导致顶层ITO温度过高致使熔断,无法进一步实现器件的完全晶化,没有达到器件仿真中预期的2Π窗口。并且为了实现器件的非晶化,需要施加高电压的窄脉冲,使相变材料达到融化温度并迅速冷却,这个过程中ITO微加热器同样会熔断,导致器件无法实现非晶化。如果要进一步实现器件的非晶化,需要改变微加热器的材料选型以及结构,比较有希望的是采用PIN微加热器的方案,由于其结构是直接形成于SOI内部的,耐用性和热稳定性更好,对于实现相变材料的非晶化问题具有很大的潜力。
使用激光器在1 540 nm~1 580 nm波长范围对光子多值器件以及直波导进行扫描,直波导所获得的光谱为光栅耦合器的光谱。因此将多值器件的光谱与光栅耦合器的光谱进行数据处理,去除光栅耦合器的影响得到器件本身的光谱特性如
3 结论
本文提出了一种基于SbSe的非易失性光子多值器件,通过有限元分析优化了器件的结构参数,使其完全相变时能够使窗口达到最大。通过在微加热器的两侧施加电脉冲来诱导晶化过程,该器件实现了超过32个态的多值调制,对非易失性光子加速器的发展具有重要意义,为构建光子智能加速器提供了一种新的解决方案。在未来通过寻找新的微加热器有望使该器件的性能进一步提高,实现功耗更低、可调状态数更多的光子多值器件。
[1] SHASTRI B J, TAIT A N, FERREIRA DE LIMA T, et al. Photonics for artificial intelligence and neuromorphic computing[J]. Nature Photonics, 2021, 15(2): 102-114.
[2] GOI E, ZHANG Q, CHEN X, et al. Perspective on photonic memristive neuromorphic computing[J]. PhotoniX, 2020, 1: 1-26.
[3] AMODEID, HERNANDEZD D. AI and compute[OL]. https://blog.openai.com/ai-and-compute/.
[5] FELDMANN J, YOUNGBLOOD N, KARPOV M, et al. Parallel convolutional processing using an integrated photonic tensor core[J]. Nature, 2021, 589(7840): 52-58.
[6] ASHTIANI F, GEERS A J, AFLATOUNI F. An on-chip photonic deep neural network for image classification[J]. Nature, 2022, 606(7914): 501-506.
[7] TAN J Y S, CHENG Z, FELDMANN J, et al. Monadic Pavlovian associative learning in a backpropagation-free photonic network[J]. Optica, 2022, 9(7): 792-802.
[8] ZHANG W, HUANG C, PENG H T, et al. Silicon microring synapses enable photonic deep learning beyond 9-bit precision[J]. Optica, 2022, 9(5): 579-584.
[9] PÉREZ D, GASULLA I, CRUDGINGTON L, et al. Multipurpose silicon photonics signal processor core[J]. Nature Communications, 2017, 8(1): 636.
[10] MARPAUNG D, YAO J, CAPMANY J. Integrated microwave photonics[J]. Nature Photonics, 2019, 13(2): 80-90.
[11] ZHU C, LU L, SHAN W, et al. Silicon integrated microwave photonic beamformer[J]. Optica, 2020, 7(9): 1162-1170.
[12] ZHOU H, ZHAO Y, WANG X, et al. Self-configuring and reconfigurable silicon photonic signal processor[J]. Acs Photonics, 2020, 7(3): 792-799.
[13] ZHENG S, LONG Y, GAO D, et al. Chip-scale reconfigurable optical full-field manipulation: enabling a compact grooming photonic signal processor[J]. ACS Photonics, 2020, 7(5): 1235-1245.
[14] XIAO X, PROIETTI R, LIU G, et al. Silicon photonic Flex-LIONS for bandwidth-reconfigurable optical interconnects[J]. IEEE Journal of Selected Topics in Quantum Electronics, 2019, 26(2): 1-10.
[15] XIE Y, SHI Y, LIU L, et al. Thermally-reconfigurable silicon photonic devices and circuits[J]. IEEE Journal of Selected Topics in Quantum Electronics, 2020, 26(5): 1-20.
[16] QIAO L, TANG W, CHU T. 32×32 silicon electro-optic switch with built-in monitors and balanced-status units[J]. Scientific Reports, 2017, 7(1): 42306.
[17] DUPUIS N, DOANY F, BUDD R A, et al. A 4×4 electrooptic silicon photonic switch fabric with net neutral insertion loss[J]. Journal of Lightwave Technology, 2019, 38(2): 178-184.
[18] WUTTIG M, BHASKARAN H, TAUBNER T. Phase-change materials for non-volatile photonic applications[J]. Nature Photonics, 2017, 11(8): 465-476.
[19] FANG Z, CHEN R, ZHENG J, et al. Non-volatile reconfigurable silicon photonics based on phase-change materials[J]. IEEE Journal of Selected Topics in Quantum Electronics, 2021, 28(3): 1-17.
[20] PARRA J, OLIVARES I, BRIMONT A, et al. Toward nonvolatile switching in silicon photonic devices[J]. Laser & Photonics Reviews, 2021, 15(6): 2000501.
[21] ZHANG Y, FOWLER C, LIANG J, et al. Electrically reconfigurable non-volatile metasurface using low-loss optical phase-change material[J]. Nature Nanotechnology, 2021, 16(6): 661-666.
[22] AGGARWAL S, MILNE T, FARMAKIDIS N, et al. Antimony as a programmable element in integrated nanophotonics[J]. Nano Letters, 2022, 22(9): 3532-3538.
[23] ZHANG H, WANG X, ZHANG W. First-principles investigation of amorphous Ge-Sb-Se-Te optical phase-change materials[J]. Optical Materials Express, 2022, 12(7): 2497-2506.
[24] CHENG Z, RÍOS C, PERNICE W H, et al. On-chip photonic synapse[J]. Advanced Science, 2017, 3: e1700160.
[25] CHENG Z, RÍOS C, YOUNGBLOOD N, et al. Device-level photonic memories and logic applications using phase‐change materials[J]. Advanced Materials, 2018, 30(32): 1802435.
[26] DELANEY M, ZEIMPEKIS I, LAWSON D, et al. A new family of ultralow loss reversible phase‐change materials for photonic integrated circuits: Sb2S3 and Sb2Se3[J]. Advanced Functional Materials, 2020, 30(36): 2002447.
[27] AMIN R, MAITI R, GUI Y, et al. Heterogeneously integrated ITO plasmonic Mach-Zehnder interferometric modulator on SOI[J]. Scientific Reports, 2021, 11(1): 1287.
宋兵, 王金融, 张亨宇, 孙振源, 李清江. 基于相变材料的非易失光子多值器件研究[J]. 光子学报, 2024, 53(1): 0123001. Bing SONG, Jinrong WANG, Hengyu ZHANG, Zhenyuan SUN, Qingjiang LI. Non-volatile Photonic Multilevel Devices Based on Phase Change Materials[J]. ACTA PHOTONICA SINICA, 2024, 53(1): 0123001.