Chiral plasmonic nanostructure of twistedly stacked nanogaps
Download: 770次
1. Introduction
Localized surface plasmons (LSPs)[1,2] are collective charge oscillations confined within the interface between the noble metal nanoparticles and the surrounding dielectric medium, and the resonance is sensitively related with the structural geometry, material, and environment[3,4]. It has been proved that LSP nanostructures with spatial asymmetry own different coupling behaviors for polarized light of opposite handedness and exhibit artificially designed chiral responses[4
Nanogap structures, such as dimers, bowties, and pairs of semicircles and rods, can confine the electromagnetic field into the narrow gaps or at the sides of particles with different incident polarizations[9,10]. Due to this polarization-sensitive resonance, the chiral responsibility of nanogap structures is studied. Until now, planar nanogap structures, including dolmen structures[11], split rings[12], and vortex gaps[13], were developed for giant chiral response.
Recently, metal–insulator–metal (MIM) three-dimensional (3D) nanostructures have attracted extensive concerns in the researches of plasmonics. It has been demonstrated that, by stacking layer by layer, the vertically aligned plasmonic structures can induce complicated charge distribution within 3D structures[1416" target="_self" style="display: inline;">–
In this work, we studied the chiral response with 3D-stacked nanogap structures. This chiral MIM nanostructure consists of two vertically and twistedly aligned nanodisks with a nanogap, resulting in hybridized charge distribution between two layers. Excited with right-handed circular-polarized (RCP) and left-handed circular-polarized (LCP) plane waves, the stacked structure twisted at 60° exhibits plasmonic coupling behaviors with/without gap modes, respectively, showing a giant chiral response (ΔT) of 60% at the wavelength of 1550 nm. This chiral response can be further tuned by controlling the particle size and the insulator layer of the structure.
2. Methodology
To understand the hybridization mechanism, plasmonic modes in nanogap structures are investigated firstly. It has been previously proved that nanogap structures own different LSP coupling performances corresponding to the alignment between the polarization and gap[10,20]. When the incident polarization is perpendicular to the gap, the near-field intensity is confined within the gap area, called as gap mode [shown in Fig. 1(a), panel (1)]. When the incident polarization is parallel with the gap, the charge distribution and the near-field intensity are located at the sides of the components, which is similar to LSP coupling behavior of single particles (SPs), named the SP mode here [shown in Fig. 1(a), panel (2)]. By twistedly stacking the two layers, the charge distribution in each gap layer is impacted and distorted by each other, leading to the hybridization of plasmonic resonance [shown in Fig. 1(a), panel (3)].
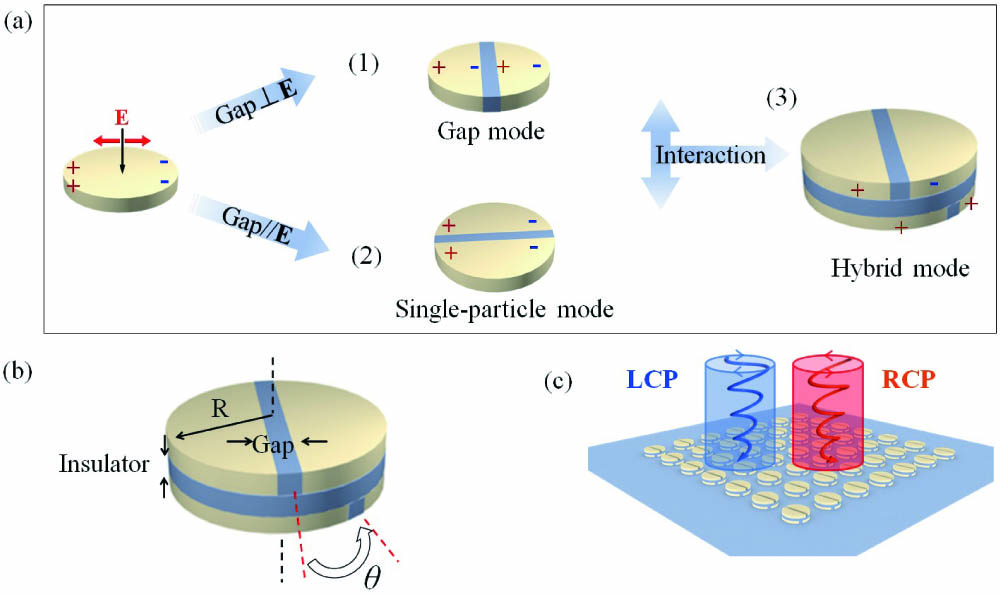
Fig. 1. Schematic diagram of structural model proposed in this study. (a) The formation of the hybrid mode. (b) The twistedly and vertically aligned MIM structure realized with the rotation of θ. (c) The proposed periodic array of the MIM structure with LCP and RCP plane waves.
Figures 1(b) and 1(c) illustrate the proposed periodic MIM nanostructure. The 3D finite-difference time-domain (FDTD) method is carried out using commercial software (FDTD Solution, Lumerical Inc.) to examine the charge distribution, near-field profile, and chiral response. For normal incidence, normalized LCP and RCP plane waves with electric field are selected to produce the chiral response, ranging from 900 nm to 2000 nm. A mesh size of 2 nm (along the , , and directions) is set to ensure the accuracy of electric/magnetic-field calculations. To optimize the circular dichroism (CD) signal at the wavelength of 1550 nm, which is one of the most important wavelengths for optical communication, the thickness and radius of the Au nanodisks with the gap size of 50 nm are set at 50 nm and 220 nm, respectively. The optical constants of Au are taken from Johnson and Christy ()[21]. Silica with the refractive index of 1.42 is selected as the substrate. The thickness of the insulator layer is set as 90 nm. The refractive indices for the surrounding medium, insulator layer, and gaps are also set as 1.42 to achieve a uniform environment for LSP coupling. The impact of the refractive index of the insulator layer is further investigated by varying the value from 1.38 to 1.46. Periodic boundary conditions in the and directions are applied with the period , and absorbing boundary conditions with a perfect matching layer (PML) are applied along the directions. The twisted angle between the two layers is varied from with a step of 10° to investigate chiral response, as illustrated in Fig. 1(b). The distributions of charge and electrical and magnetic near-field are further investigated with varied twisted angles, and the corresponding chiral response is valued by CD[22]:
3. Results and Discussion
The transmission spectra for LCP and RCP plane waves with different twisted angles are investigated firstly. As shown in Figs. 2(a) and 2(b), the LCP and RCP transmission spectra are the same for the twisted angle at 0° and 90°, since there is no structural asymmetry for these structures. For the twisted angle at 60°, the absorption peak around 1550 nm [marked in Fig. 2(a)] is discovered in the transmission spectrum for the LCP plane wave, and the transmittance is only 21.3%. In contrast, the transmittance for the RCP plane wave at the same wavelength is 83.5%. The transmission difference proves that there is a giant chiral response for this twisted MIM nanostructure.

Fig. 2. Transmission spectra of MIM structures for (a) LCP and (b) RCP plane waves with different twisted angles at 0o, 30o, 60o, and 90o, respectively. (c) The detailed transmission spectra of the MIM structure with twisted angles varied from 90o to 0o with a step of 10° for LCP and RCP plane waves, respectively. The radius of the nanodisks is 220 nm, and the gap in the disk is 50 nm.
Figure 2(c) illustrates the trends (marked as yellow dash lines) of transmission by twisting the structure in details. For the LCP plane wave, the absorption peak is red-shifted originally from 1152 nm (marked as red star) to 1785 nm with decreased from 90° to 0°. Meanwhile, the same absorption for the RCP plane wave is inverted to enhanced transmission with decreased . The giant transmission difference with the twisted angle at 60° at the wavelength of 1550 nm is noted as green stars in Fig. 2(c). Calculated CD signals () impacted by varied structural parameters are concluded in Fig. S1 of Supplementary Material. Absorption spectra also perform chiral responsivity, shown in Fig. S2 of Supplementary Material.
The transmission difference indicates that the chiral response is impacted by the twisted angle. Correspondingly, charge distributions of the MIM structures twisted from 0° to 90° are analyzed at the wavelength of 1550 nm. The results with the twisted angle of 0°, 30°, 60°, and 90° are shown in Figs. 3(a)–3(d), respectively. For easy understanding, simplified charge oscillations with the same conditions are illustrated in Figs. 3(e)–3(h). With the twisted angle at 0°, the charge distributions of both LCP and RCP excitations are located at the sides of the particles, showing the typical SP mode [shown in Figs. 3(a) and 3(e)]. With the twisted angle at 90°, the SP mode exists in the bottom layer, and the gap mode exists in the top layer for both LCP and RCP excitations [shown in Figs. 3(b) and 3(f)]. By increasing from 0° to 30°, the SP mode still exists in the top layer, but the intensity of charge distribution for the RCP condition is weakened, causing the difference of the LSP coupling effect and transmittance [shown in Figs. 3(c) and 3(g)]. Noticeably, for at 60°, the top layer for the LCP plane wave shows the SP mode, while for the RCP condition it diversely shows the gap mode [shown in Fig. 3(d) and marked in the inset of Fig. 3(h)]. For the LCP condition, the charge distribution of the two layers is close to an antibonding-type coupling between two layers [marked in Fig. 3(d)], which is previously convinced in MIM structures[23], while the SP mode of the bottom layer for the RCP condition performs ambiguously with lower intensity. Thus, the antibonding-type coupling for the RCP condition is much weaker than that for the LCP condition, leading to lower absorption and higher transmittance. Corresponding to this difference in coupling modes, the maximum transmission difference achieves 60% for at 60°.
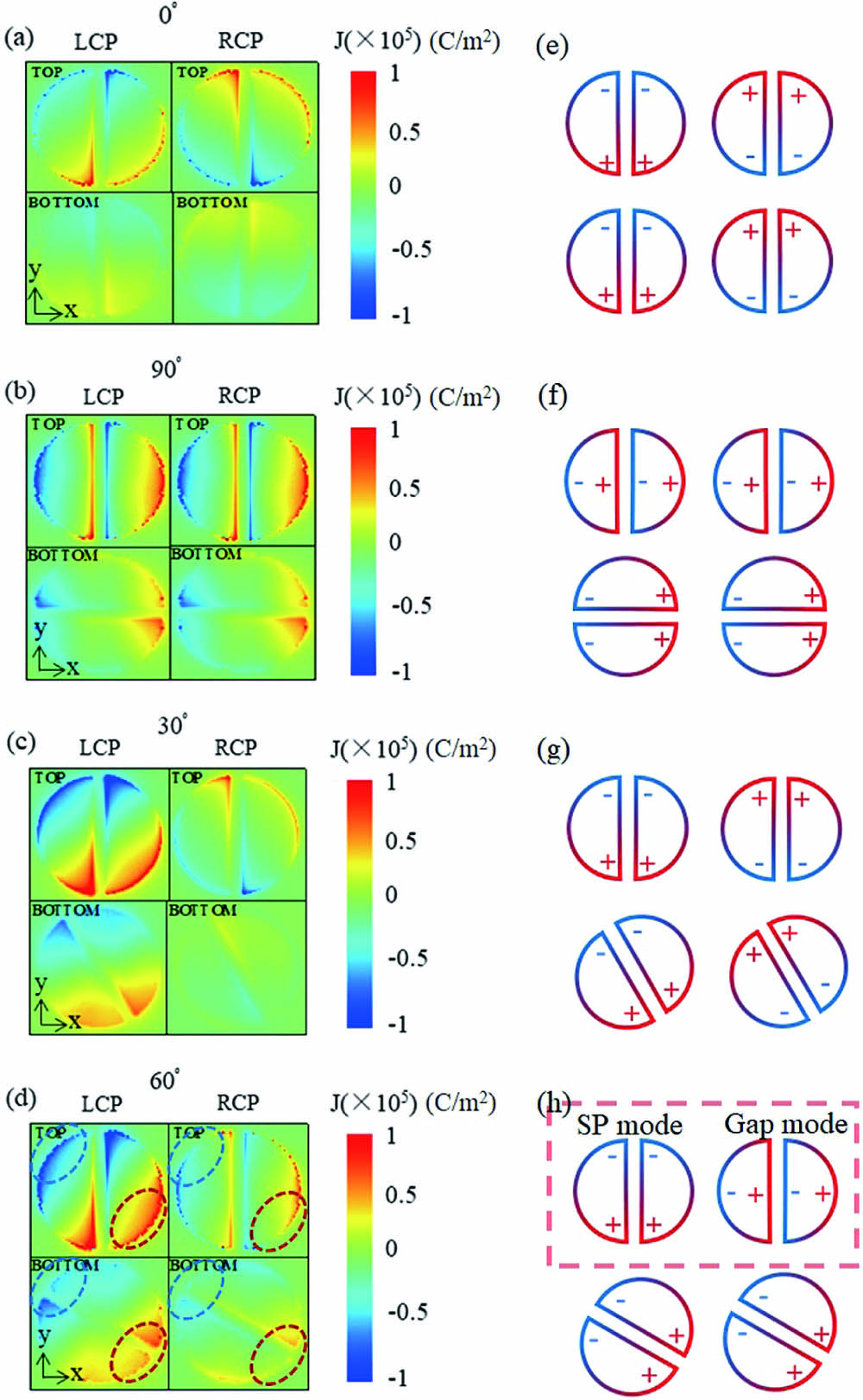
Fig. 3. Simulated charge distributions of the top and bottom nanogaps twistedly stacked with (a) 0o, (b) 90o, (c) 30o, and (d) 60o, excited with LCP and RCP plane waves at the wavelength of 1550 nm, respectively. Simple drawing for charge oscillations of the same structures with the twisted angle of (e) 0o, (f) 90o, (g) 30o, and (h) 60o, excited with LCP and RCP plane waves at the wavelength of 1550 nm, respectively. Inset in (h): SP mode and gap mode for the same structure with LCP and RCP plane waves, respectively.
The optical chirality for monochromatic electromagnetic radiation in free space is expressed as[24]

Fig. 4. Calculated magnetic near-field distributions of top and bottom nanogaps twistedly stacked with (a) 0°, (b) 90°, (c) 30°, and (d) 60°, excited with LCP and RCP plane waves at the wavelength of 1550 nm, respectively.

Fig. 5. CD signals of the MIM structure impacted by twisted angles varied from 0° to 90°. (a) T spectrum for structures twisted from 0° to 90° with a step of 10°. The maximum CD signal is achieved with the twisted angle of 60°, as the red arrow marks. (b) Grayscale image of the CD signal ( T) impacted by the twisted angle and plane wave wavelength. The maximum CD signal is achieved at 1550 nm for the twisted angle of 60°, as the green star marks.
Finally, the tunability of chiral behavior of this structure is investigated. The size of the nanodisk impacts the chiral response, as shown in Fig. 6(a). For the structure twisted at 60°, the maximum CD signal () shows red shift from 1538 to 1626 nm by increasing the radius of the nanodisk from 130 nm to 260 nm. The impact of the refractive index of the insulator layer is also investigated in Fig. 6(b). The chiral response can be tuned from 1538 nm to 1626 nm when the increases from 1.38 to 1.46, showing critical sensitivity to the change of the medium index.

Fig. 6. (a) Maximum of CD ( T) with varied Au nanodisk radius from 120 nm to 250 nm, in which the red shift with the increasing nanodisk radius is marked by the dash line. (b) Maximum of CD ( T) with varied refractive index from 1.38 to 1.46, in which the red shift with the increasing refractive index is marked by the dash line.
4. Conclusions
We theoretically study the chiral response of twistedly stacked nanogap structures. With the twisted angle of 60°, LSP coupling performance with/without gap modes for RCP and LCP excitation at 1550 nm is discovered. The electrical and magnetic near-field distributions of this twisted MIM structure corresponding to different polarizations are further validated. As a result, giant chiral response () at the wavelength of 1550 nm is realized, which shows critical sensitivity to the change of the nanodisk size and the medium index. Our result reveals the hybridization of LSP coupling behavior in twistedly stacked nanogap structures, which perform as a kind of ideal chiral metamaterial.
[16] M. Hentschel, M. Schäferling, X. Duan, H. Giessen, N. Liu. Controlling metamaterial transparency with superchiral fields. Sci. Adv., 2017, 3: e1602735.
[24] K. Y. Bliokh, F. Nori. Characterizing optical chirality. Phys. Rev. A, 2011, 83: 021803.
Jian Zhang, Rui Tu, Chao Huang, Xiaoli Yao, Xin Hu, Haixiong Ge, Xuefeng Zhang. Chiral plasmonic nanostructure of twistedly stacked nanogaps[J]. Chinese Optics Letters, 2021, 19(1): 013601.